Single-Step Wet Synthesis of Copper Oxide Nanoparticles, Characterization and their Biological Activities
Received Date:March 24, 2020Accepted Date:April 09, 2020Published Date:April 12, 2020
doi: 10.17303/jmsa.2020.4.103
Citation:Sania Naz (2020) Single-Step Wet Synthesis of Copper Oxide Nanoparticles, Characterization and their Biological Activities. J Mater sci Appl 4: 1-11.
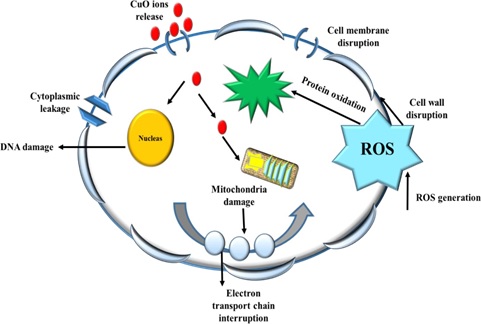
Abstract
This work demonstrates an efficient and facile synthesis of copper oxide nanoparticles via a single-step chemical wet process. Using UV-visible spectrophotometry (UV-vis), X-rays Diffraction (XRD), and Fourier transform infrared (FTIR) spectroscopy, optical, structural and compositional analysis of CuO NPs were investigated. Results reveal the successful fabrication of pure phase monoclinic crystalline structures with an average diameter of 32.85 nm. To elucidate the biological activities of prepared nanoparticles, antioxidant, phytochemical, antimicrobial, enzymatic inhibitions and brine shrimp lethality assay were performed. Minimalist antioxidant and photochemical behavior were elucidated following by moderate cytotoxic potential of CuO nanoparticles. Furthermore, CuO NPs exhibited well antimicrobial activity against a wide range of micro-organisms such as E. coli, Staphylococcus aureus, Pseudomonas aeruginosa, and Bacillus substiles and fungal species i.e., Mucorspecies, Aspergillus niger, Aspergillus fumigatus and Fusarium solani. The morphological and compositional properties along with excellent antimicrobial performance make it valuable for routine practices in water treatment and medical technology.
Keywords: CuO NPs; Monoclinic crystalline; Brine shrimp lethality; Antimicrobial
Introduction
Nanotechnology encompasses the tailoring of materials at the nano-scale to achieve distinctive properties that can be manipulated for desired applications in the industrial, environmental and health sectors [1]. The engineered nanoparticles exhibit novel physical, chemical and biological characteristics. Metal oxide nanoparticles (NPs) attain significant attention for their potential applications in nanodevices, nanoelectronics, optoelectronics, nanosensors, and catalysis. Amid different metal oxide NPs, CuO nanoparticles have attracted particular consideration owing to its interesting properties i.e., is the simplest member of copper compounds family, a p-type semiconductor with a narrow band gap (1.2 eV in bulk) which displays a range of expedient physical properties like high thermal and superconductivity, spin dynamics and electron correlation effects.
CuO NPs are progressively used in several applications such as in catalysis, magnetic storage media, super capacitors, near-infrared filters, solar energy, batteries, gas sensors, heat transfer fluids, and in the preparation of various organic-inorganic nanocomposites [2]. CuO nanomaterials can be potentially used in MRI-ultrasound dual imaging contrast agents. They provide radiation-free high spatial resolution scans by MRI and low cost high temporal resolution by ultrasound [3]. The copper nanoparticles is effective on fluorescent materials and hence may cause fluorescence enhancement, aggregation of dyes, and fluorescence quenching. This feature might be useful for bio-labeling and bio-sensing. Copper-based drugs are enormously used to destabilize/weaken cancer cells and tumors. In hemoglobinopathies, they act as a screening agent for the diagnosis of b-thalassemia as the clumps precipitate with human hemoglobin (Hb) variant. High anti-thrombin activity and imaging routine applications of CuO NPs have been explored recently. Water pollution is a global threat to human health being contaminated by microorganisms, CuO NPs have been used as a disinfectant and antifouling agent in wastewater treatment [4, 5]. As per literature, the photocatalytic activity of CuO NPs has been reported against several dyes viz., bromophenol blue, Nile blue, reactive yellow and other textile dyes [6-8].
Different synthetic techniques are adopted to synthesize copper oxide nanoparticles (CuO NPs) with distinguished morphological characteristics i.e., chemical, physical and biological approaches. The physical method viz., laser ablation involves no use of capping or reducing agents and synthesizes ellipsoidal shape CuO NPs. The biological technique utilizes sources of bacteria, algae, fungi, and plants to synthesize CuO NPs of various sizes and shapes. The disadvantages of former techniques are low yield, a requirement of higher energy, tedious down streaming procedures, time-consuming, laborious, and pathogenicity risk in fungi modulated synthesis. Amongst all the available approaches, chemical methods (chemical reduction, precipitation, electrochemical deposition, sonochemical, hydrothermal, ultra-sonication, sol-gel) are the utmost explored routes for the synthesis of CuO NPs. Their advantages are cost-effectiveness, less laborious, high yield, controlled NPs size and shape, fast and clean [9].
Addressing the importance of copper oxide nanoparticles and their diverse applications, the present work reported the synthesis, characterization and different biological activities of CuO NPs. A successful synthesis of CuO NPs was attained by a wet chemical technique as performed previously with few modifications [10]. The formation of CuO nanoparticles was confirmed by UV-visible, XRD and FTIR spectroscopic techniques.
Materials and Methods
Reagents and Chemicals
All the chemicals used in these experiments were of analytical grade. Laboratory glassware was kept overnight in a 10% (v/v) HNO3 solution and then rinsed with deionized water. The ISP4 medium (Difco Laboratories), TSB medium (Sigma-Aldrich), Luria broth and SDA (Oxide) were used as a medium in biological assays. The deionized water and distilled water were used throughout the experiment as a solvent.
Synthesis of CuO NPS
A simple wet method was used for the synthesis of CuO NPs. Briefly, the aqueous solution of precursor salt of copper (copper acetate monohydrate) was preheated and stirred continuously. Followed by the dropwise addition of sodium hydroxide and allowed this reaction for 3 hr under continuous stirring. After completion of the reaction, NPs were isolated via centrifugation at 10,000 rpm for 10 min and washed thrice with distilled water. Pellets were dried in a hot air oven at 60 ºC overnight and calcined at 450 ºC. The as-prepared CuO NPs were used as it for characterization and biological activities.
Characterization
Different characterization techniques were employed to explore the morphological properties (size, shape, surface area, dispersity) of synthesized copper oxide nanoparticles. The ultraviolet-visible spectrometer (Shamazo UV-Vis spectrophotometer) was used to obtain the optical properties, X-ray diffraction (X-Pert XRD) for crystal structure characterization, phase identification and crystallite size, Fourier transforms infrared spectroscopy (Bruker FTIR) explore the surface chemistry and organic functional groups attached on the surface of CuO NPs.
Antioxidant Assay
To determine the antioxidant potential of chemically synthesized copper oxide nanoparticles following assays were performed i.e. total antioxidant capacity determination (TAC), DPPH free radical scavenging assay and total reducing power assay (TRP).
Total Antioxidant Capacity (TAC)
The total antioxidant capacity of synthesized CuO NPs was ascertained by Phospho-molybdenum based protocol [11]. Briefly, a 100μl aliquot of sample was allowed to react with 900μl of reagent (0.6 M sulphuric acid, 28 mM sodium phosphate and 4 mM ammonium molybdate). The mixture was heated in a water bath for 90 min at 95ºC. After the mixture was cooled down 20μl sample was transferred to 96 well plates to measure the optical absorbance at 630 nm. The calibration curve (y = 0.057x + 0.748, R2 = 0.99) was drawn in accordance to the absorbance of positive control i.e. Ascorbic acid at final concentrations 50, 25, 12.5 and 6.25μg/ml under the same experimental conditions. The antioxidant activity is expressed as the number of μg equivalents of ascorbic acid per milligram sample i.e., μg AAE/mg sample [12].
Total Reducing Power (TRP)
Total reducing power of a compound plays a major role in neutralizing and absorbing free radicals. The capability of an antioxidant to donate an electron can be measured by reducing power assay [13]. To determine the reducing power of copper oxide nanoparticles the following protocol was used [14, 15]. From 4 g/ml stock solution, a 200 μl was transferred into Eppendorf tubes and was uniformly mixed with 200 μl0.2 M phosphate buffer (pH 6.6) and 250μl 1% potassium ferricyanide. The tubes were incubated for 20 min at 50 ºC and 200 ml of 10% Trichloroacetic acid was added to each tube. The tubes were centrifuged at 3000 rpm for 10 min. A 150 μl of supernatant was transferred into 96 well-plates and 50 μl of 0.1% Ferric chloride was added in each well. Optical absorbance was recorded at 630 nm. The calibration curve (y = 0.014x + 0.891, R2 = 0.998) was drawn in accordance with the absorbance of positive control i.e. Ascorbic acid at final concentrations of 100, 50, 25 and 12.5 μg/ml under the same conditions. The resultant reducing power of each sample is expressed as μg AAE/mg DW.
Free radical scavenging activity-DPPH
To elucidate the antioxidant potential of CuO NPs, 10 μl of each sample (4 mg/ ml) was treated with 190 ml of DPPH reagent (9.6 mg/ 100 ml methanol) in 96 well plates following the previous protocol [16, 17]. The reaction mixtures were incubated at 37ºC for 1 hr and optical absorbance was recorded at 517 nm. Ascorbic acid and DMSO were used as a positive and negative control, correspondingly. To calculate the scavenging activity in percent (%RSA) following equation was used:
Percent radical scavenging capacity = (1 – Abs / Abc) *100 Where;
Abs represents the absorbance of the DPPH solution with a sample.
Abc indicates the absorbance of the negative control (containing the reagent as a substitute for sample).
Flavonoid like activity
According to the system suitability, the aluminum chloride colorimetric method with slight modifications was used for total flavonoid content determination [18]. A 20μl of the test samples (4 mg/ml DMSO), blank, positive controls, and negative control were added into each well of 96 well microplates. Subsequently, 10 μl of 1.0 M potassium acetate and 10% aluminum chloride was added into each well. This was followed by the addition of 160 μl of distilled water to make up the final volume up to 200 μl. The plate was then incubated at room temperature for 30 min. The optical density of the plate was measured at 415 nm using a microplate reader. At final concentrations (2.5, 5, 10, 15, 20 and 40 μg/ml) the calibration curve was drawn by using quercetin as a standard. By using the equation y = 0.0368x + 1.0954(R2 = 0.9872) calibration curve of quercetin was drawn. The assay was performed in triplicate and the resultant flavonoid content was recognized in μg equivalents of quercetin per milligram sample (μg QE/mg sample).
Phenolic like activity
An aliquot of 20 μl of test samples, positive control (Gallic acid), negative control (DMSO) and blank were taken into 96 well plates [19]. In each well, 90 μl Folin-Ciocalteu reagents were added and microplate was incubated at room temperature for 5 min. After incubation, 90 μl of sodium carbonate was added into the reaction mixture in each well. Gallic acid was used as a standard and optical density of reaction mixture was recorded at 630 nm using a microplate reader (Biotech USA, microplate reader Elx 800). A calibration curve (y = 0.102x -0.3048, R2 = 0.9889) was obtained. This assay was performed in triplicate and the results were expressed as μg Gallic acid equivalent per milligram sample (μg GAE/mg sample).
Antimicrobial Assay of Copper Oxide Nanoparticles (CuONPs)
The antimicrobial assay was executed by using the "Disc diffusion method" to explore the antimicrobial potential of chemically synthesized copper oxide nanoparticles.
Antibacterial Assay
The antibacterial properties of synthesized CuO NPs (4 mg/ml) were evaluated by using the disc diffusion assay against five bacterial strains E. coli, Bacillus subtilis, Staphylococcus aureus, Pseudomonas aeruginosa, and Klebsiella pneumonia. Rothrimycine antibiotic was used as a positive control for this assay while disc infused with DMSO was used as a negative control. The 5 μl of each sample (4 mg/ml) was poured on sterile filter paper discs previously infused on the cultured plates. A 5 μl of Roxithromycin and 5 μl of DMSO was poured on separate discs which served as positive and negative controls respectively. The plates were incubated for 24h at 37ºC thereafter average diameter of zone of inhibition was measured [11].
Antifungal Assay
Antifungal activity of CuO NPs was monitored by using five antifungal strains namely, Mucor species (FCBP-0300), Aspergillus flavus (FCBP-0064), Aspergillus niger (FCBP-0198), Aspergillus fumigatus (FCBP-66), and Fusarium solani (FCBP-0291) species. The Disc diffusion method was used for antifungal activity analysis. The SDA media (Sabouraud dextrose agar, pH 5.7) was autoclaved and poured in Petri plates under sterile conditions. Fungal lawns were prepared by inoculating spores on the surface of the media after that disc loaded with 5 μl of 4 mg/ml sample were placed on the surface of the Petri plate. The plates were incubated at 25°C for 1 day and the zone of inhibition was measured, respectively [11].
Cytotoxicity (Brine Shrimp Lethality Assay)
Cytotoxic potential of chemically synthesized CuO NPs was examined by brine shrimp lethality assay as described previously [20, 21]. The brine shrimp lethality bioassay is largely used in the evaluation of nanomaterials toxicity. This assay offers a competent preliminary approach to govern anti-tumor, anti-microbial, anti-malarial and insecticidal activities. Samples with a final concentration of 25, 50, 100 & 200 μg/ml were used. Brine shrimp (Artemia salina) eggs were hatched in artificial seawater (prepared by dissolving 38 g/L sea salt in water) and were incubated for 48 h at 37 °C. After 2 days nauplii were transferred with Pasteur pipette in the vials containing 2 ml of sample. Ten larvae were transferred to each well and seawater was added to make the volume up to 300μl. A vial containing 50 μl DMSO instead of the sample was used as control. After 24 h of incubation, the number of survived shrimps were counted and recorded in each vial. Etoposide was used as a positive control. Final data were analyzed with a Finnery computer program to determine the LD50 values with a 95% confidence level.
Protein Kinase Inhibition Assay
Protein kinase inhibition assay was performed by following a previous procedure [11]. The ISP4 medium was used for the production of Streptomyces (largest genus of Actino- bacteria) spores while liquid TSB medium was used for mycelium propagation. Streptomyces culture was refreshed on TSB medium in a shaker incubator at 28°C. An aliquot of 60 μl of refreshed culture was taken in an Eppendorf tube and mixed with 540 μl of sterile TSB media. The ISP4 medium was autoclaved and 25 ml was poured into sterile Petri plates and allowed to solidify. Sterile cotton swabs were used to culture inoculum homogeneously over the entire surface of the Petri plates softly. The discs of 6 mm diameter were placed on the surface of the Petri plate along with the 5μl of CuO NPs (4 mg/ml in DMSO) and negative control (DMSO) was poured carefully on the discs. After incubation of plates at 37°C for 24 h; the diameter of the zone of inhibition was measured.
Alpha-amylase inhibition assay
Chemically prepared CuO NPs were utilized for alpha-amylase activity according to the method described earlier [22]. In dis. H2O 0.5% w/v potato starch is used. The solution for the enzyme was made by the dissolution of α-amylase in 20mM sodium phosphate buffer comprising 6.7mM NaCl. Various concentrations of CuO NPs were made in DMSO. DNS reagent was prepared by combining 5.31M sodium potassium tartrate, 96mM 3, 5- dinitro salicylic acid, 2M NaOH, and distilled water. In a solution, 500 μl enzyme mixture and 500 μl CuO NPs were added. From the mixture, 500 μl was taken off and mix with 500 μl potato starch solution. After that, 500 μl DNS reagent was added and the mixture was allowed to keep at 85 ºC in a water bath for 10 min duration. The reaction solution was diluted with distilled water (4.5ml) and at 540 nm wavelength absorbance value was measured. For blank, the color reagent was added before starch solution whilst in control, 500 μl DMSO and 500 μl enzyme solution was added. At 100-500 μg/ml concentrations, antidiabetic drug acarbose was utilized as a positive control.
Results
Synthesis of CuO NPs
The successful synthesis of CuO NPs was achieved by a wet chemical method. Immediate change of color obtained after the addition of NaOH which confirmed the synthesis of CuO NPs.
Characterization
The synthesis of CuO NPs was further confirmed by the UV Vis spectroscopy. A broad and intense absorption peak was observed around 370 nm verifying the successful synthesis of CuO NPs as displayed in (Figure S1).
The XRD pattern of CuO NPs synthesized through a simple wet chemical method was described in (Figure S2). All diffraction peaks obtained were consistent with the pure monoclinic CuO NPs with the JCPD card 05-0661. The phase pure CuO NPs with monoclinic geometry were successfully synthesized with no characteristic peak of any impurities. The crystalline size of as-prepared CuO NPs was 32.85 nm calculated by the Scherrer's equation.
The (Figure S3) illustrated the FTIR spectrum of CuO NPs. Two weak absorption peaks were seen at 3410 and 1640 cm-1 corresponds to the O–H stretching because of the presence of moisture on CuO NPs surface and carboxylic group. Two prominent peaks at 598 and 478 cm-1 attributed to Cu-O stretching of CuO NPs. These results verified the successful synthesis of CuO NPs with monoclinic geometry.
Antioxidant activities
Figure 1 compares the antioxidant and the reducing potential of synthesized CuO NPs. The TAC displays the quenching capability of examined compounds towards ROS production. The total antioxidant capacity (TAC) depends on the reduction of Mo (VI) to Mo (V) via antioxidant mediators and as a result, Phosphate/Mo (V) complex (Green in Color) is formed with maximal absorption at 695 nm. To further examine the occurrence of antioxidant species reducing power assay was performed. This involves the use of reductones that are linked with antioxidant potential by contributing H-atoms, which lead towards damaging of the free radical chains. TAC and TRP activities of CuO NPs were calculated and expressed as Ascorbic acid equivalent (μg AAE /mg sample). The results elucidated the highest reducing potential whilst the comparatively low antioxidant potential of copper oxide nanoparticles as 20.2 and 18.5 AAE /mg respectively.
The percent free radical scavenging activity (RSA) of the copper oxide nanoparticles was elucidated by the discoloration of the DPPH reagent. This technique depends on the reduction of DPPH (Purple in Color) into stable diphenyl picrylhydrazine molecule (Yellow in Color) by accepting hydrogen radical or electron from the donor antioxidant. The copper oxide nanoparticles showed some radical scavenging activity (14.0%).
Phytochemical analysis
Phenols and flavonoids are bioactive compounds of plants. These are very important secondary metabolites [25]. So, it is obvious that these compounds will be in higher concentration in plant extracts as compared to nanoparticles. As Folin-Ciocalteu assay is sensitive to phenols that's why it is used for the determination of phenolic content. Gallic acid equivalents (μg (GAE)/mg sample) are used to represent TPC while Quercetin equivalent (μg QE/mg sample) expresses TFC.
To explore the free radical scavenging activity and antioxidative potential of nanoparticles, phenolic and flavonoid like the potential of nanoparticles were investigated (Figure 1). The copper oxide nanoparticles demonstrated different behavior in response to reaction mixtures for phenolics and flavonoids. These activities are most presumably due to the presence of different functional groups on the surface of the nanoparticles. Copper ions released from the copper oxide NPs might also be involved in these activities. In nanoparticles TFC and TPC are very less to negligible i.e. TFC values, (4.1 μg QE/mg sample) and TPC values are (17.5 μg GAE/mg sample) of CuO nanoparticles.
Enzyme Inhibition Activities
In the current study, no protein kinase inhibition activity was observed. Protein kinases are the enzymes responsible for different nucleotide residues phosphorylation, which serves as an important regulatory process for the metabolism. Surfactin was used as a positive control and revealed a 17 mm bald zone in comparison to CuO NPs. This indicates that chemically synthesized CuO NPs exhibit no protein kinase inhibitory potential.
Moreover, chemically synthesized CuO NPs were elucidated for alpha-amylase inhibition activity. The function of alpha-amylase is to convert the carbohydrates into glucose, henceforth, hindering the activity of alpha-amylase can inhibit the glucose level which is an integral part of diabetes research [26]. Our results found that CuO NPs showed mild alpha-amylase inhibition that is 15.6% (Figure 1).
Antibacterial activity
(Figure 2) indicates the antibacterial activity of copper oxide nanoparticles (CuO NPs) against five bacterial strains namely, Escherichia coli (ATCC 15,224), Bacillus subtilis (ATCC# 6633), Staphylococcus aureus ((ATCC# 6538), Pseudomonas aeruginosa ((ATCC# 9721) and Klebsiella pneumonia ((ATCC# 4619) adapting the agar well diffusion method. The bigger zone of inhibition signifies more active antimicrobial compounds. Copper oxide nanoparticles showed a variety of responses against different strains of bacteria in the form of zones of inhibition of different diameters. The results show that copper oxide nanoparticles exhibit better activity against Bacillus specie and also give significant performance against other bacterial strains.
The copper oxide nanoparticles exhibited potent antibacterial activity with an inhibition zone of 9 mm against Bacillus subtilis, whereas against K. pneumonia lowest activity was shown. Similarly, in the case of E. coli and P. aeruginosa, antibacterial activity was observed as an 8 mm zone of inhibition whilst 7mm zone of inhibition was measured by S. aureus respectively.
Anti-fungal activity
For antibacterial evaluations, metal-based nanoparticles have been repeatedly used whilst less consideration has been given to antifungal properties. In this work, the antifungal efficacy of chemically synthesized CuO NPs was identified using the disc diffusion method. Antifungal potential of CuO NPs is evidenced against all tested strains; Mucor species (FCBP-0300), Aspergillus flavus ((FCBP: 0064), Aspergillus niger (FCBP: 0918), Aspergillus fumigatus (FCBP: 66), and Fusarium solani species (FCBP: 0300). DMSO was used as a solvent. Amp B (250 μg/ml) was used as a positive control. Different antifungal activity was observed against all strains. Among the tested strains, the maximum zone of inhibition exhibited by solani is 13mm following by Mucor, fumigatus, and niger correspondingly as shown in (Figure 3). The Cu ONPs were found ineffective against Aspergillus flavus species.
Brine Shrimp Lethality Assay of Cu ONPs
Brine shrimp lethality assay is performed to evaluate the cytotoxic potential of copper oxide nanoparticles at concentrations 200, 100, 50, 25μg/ml, respectively. It is observed that percentage mortality decrease with the decrease of the nanoparticle concentration. The highest inhibition is reported at 200 μg/ml and 100 μg/ml i.e. 80 and 60% respectively. The LC50 value obtained is 70μg/mL, correspondingly. The mortality rates of the test samples are shown in (Figure 4).
Discussion
In this work, the chemical route has opted for CuONPs synthesis and their morphological characterizations, biological activities and phytochemical analysis was performed. Owing to the flexible properties of copper oxide nanoparticles i.e., large surface to volume ratio, high thermal and electrical conductivity have increased their usage in potential applications viz, electronic devices, super capacitors, fuel cells, sensors, industrial catalyst, biomedicines, and bioremediation. In the literature, successful chemical synthesis of copper oxide nanoparticles has been evidenced [27,28] by a change in the color [10, 23]. UV-vis and XRD analysis confirmed the synthesis of CuO NPs. The Cu ONPs exhibited characteristic SPR peak at 370 nm which is in accordance with the previous studies [24, 29, 30]. CuONPs are direct band gap materials, upon receiving photon energy electronic transitions take place from the valence to conduction band that results in absorption. According to the reports, the band gap in response to antibacterial activity gives information on energy efficacy towards electrons-holes production in conduction and valence band [31, 32]. With bacterial species, the electrons-holes pairs interact and results in the production of reactive oxygen species (ROS) leading towards the disruption of living cells. Furthermore, for metal oxide nanoparticles wide range of spectrum ranging from UV to visible light is common. Although, their maximum peak took place in the UV region which signifies that NPs have a variant range of physical morphology (size and shape) [33, 34]. The XRD analysis confirmed that the synthesized CuONPs are fine and pure. All diffraction peaks reveal the good crystalline nature of as-synthesized material. The results are in good agreement with the previous reports indicating the monoclinic phase of CuONPs [35, 36]. In the FTIR spectrum of CuONPs, there were two weak peaks at 3410 and 1640 cm-1 corresponds to the O–H stretching because of the presence of moisture on the surface of CuONPs and the carboxylic group. Two prominent peaks at 598 and 478 cm-1 attributed to Cu-O stretching of CuONPs. These results verified the successful synthesis of CuONPs with monoclinic geometry. These findings were according to the previous reports [35, 37-39].
The insignificant antioxidant activities of chemically synthesized CuONPS as compared to a green route are due to a lack of capping agents as their purity revealed from XRD analysis. There are no additional functional groups attached to the surface of nanoparticles. From the present work, it is clearly evident that antioxidant potential not only depends on the capping agents but also accredited to other parameters such as the method used in the synthesis NPs, surface charge, size & shape and other chemical properties [40]. The phytochemical analysis of CuONPs exhibited distinct behavior in response to phenolics and flavonoids reaction mixtures. Enzyme inhibition of nanoparticles has been rarely discussed. The enzymatic activities of synthesized CuONPs viz., protein kinase and alpha-kinase inhibitions were elucidated. In the present study, no protein kinase inhibition activity of CuONPs was observed whilst performed 15.6% alpha-amylase inhibition as compared to other reports [16, 41, 42].
Metal oxide nanoparticles displayed different antimicrobial activity against the gram –ve and gram +ve bacterial species. The antimicrobial mechanism of copper oxide nanoparticles has been suggested by many researchers [43, 44]. The generation of reactive oxygen species (ROS) is considered as one of the primary sources. Overall, CuO NPs showed significant antibacterial action on both classes of bacteria, which may be attributed to the large abundance of amines and carboxyl groups on their cell surface and greater affinity of copper ions toward these groups. The antibacterial reactivity of copper oxide nanoparticles is attributed due to their large surface area which enables them to interact with the bacterial cell membranes. Upon penetration, the copper ions interact with the DNA molecule and disrupt biochemical processes. Previous reports validated the antibacterial activity of copper oxide nanoparticles due to the Cu ion release and ROS induction. The copper ions released from copper oxide nanoparticles inhibit the cellular mechanism of bacterial growth as displayed in (Figure 5) [45]. After exposure to CuONPs, ROS induction takes place following DNA damage and subsequent cell death [46]. The antibacterial results are consistent with the previous findings [15, 40,47-52].
In addition to reactive oxygen species generation (ROS), CuONPs exhibit moderate antifungal activities by interfering with fungal hype and fungal spores leading towards inhibition of fungal growth. Our findings were well supported and in agreement with available literature [53, 54]. To evaluate the cytotoxic potential of synthesized CuO NPs, brine shrimp lethality test was conducted. The nanoparticles gave maximum inhibition that was in agreement with the reports as smaller nanoparticles induce a higher level of cytotoxicity [55].
Conclusion
The present study demonstrates the efficient and facile synthesis of copper oxide nanoparticles through chemical route. The synthesized nanoparticles were characterized by using UV-visible spectrometry, XRD and FTIR. The nanoparticles showed minimalist behavior of antioxidant, enzymatic inhibition and phytochemical characteristics. Moderate cytotoxic property of CuONPs was also shown. Moreover, CuONPs exhibited well antimicrobial potential against a wide range of gram-positive and gram-negative bacterial and fungal strains. From the observed and well-reported antimicrobial properties of copper oxide nanoparticles, it can be concluded that further research should be executed towards the decoration of chemically synthesized CuONPs on supporting materials like MOFs, cotton, polymers, filtration membrane to explore their wider applications in the wastewater treatment. Nanoparticles toxicology is an utmost aspect, therefore to establish and confirm their usage safety, in vivo studies are highly recommended.
- Lee YC and JY Moon (2020) Introduction to Nanotechnology and Bionanotechnology. An Introduction to Bionanotechnology Springer, Singapore 1-14.
- Ahamed M, et al. (2014) Synthesis, characterization, and antimicrobial activity of copper oxide nanoparticles. Journal of Nanomaterials.
- Perlman O, IS Weitz, and H Azhari (2015) Copper oxide nanoparticles as contrast agents for MRI and ultrasound dual-modality imaging. Physics in Medicine & Biology 60: 5767.
- Bhattacharya P, et al. (2019) Disinfection of drinking water via algae mediated green synthesized copper oxide nanoparticles and its toxicity evaluation. Journal of Environmental Chemical Engineering 7: 102867.
- Saraswathi MSSA, et al. (2020) Versatility of hydrophilic and antifouling PVDF ultrafiltration membranes tailored with polyhexanide coated copper oxide nanoparticles. Polymer Testing 106367.
- Rashad M and HAAl-Aoh (2019) Promising adsorption studies of bromophenol blue using copper oxide nanoparticles. Desalination and Water Treatment 139: 360-368.
- Singh J, et al. (2019) Biogenic synthesis of copper oxide nanoparticles using plant extract and its prodigious potential for photocatalytic degradation of dyes. Environmental Research 177: 108569.
- Rao MP, et al. (2018) Simple and low-cost synthesis of CuO nanosheets for visible-light-driven photocatalytic degradation of textile dyes. Journal of Environmental Chemical Engineering 6: 2003-2010.
- Jillani S, et al. (2018) Synthesis, characterization and biological studies of copper oxide nanostructures. Materials Research Express 5: 045006.
- Naz S, et al. (2017) Kinnow peel extract as a reducing and capping agent for the fabrication of silver NPs and their biological applications. IET nanobiotechnology11: 1040-1045.
- Zia M, et al. (2016) Green synthesis of silver nanoparticles from grape and tomato juices and evaluation of biological activities. IET nano biotechnology 11: 193-199.
- Clarke D, et al. (2013) Detection and learning of floral electric fields by bumblebees. Science 340: 66-69.
- Alavi M and N Karimi (2018) Characterization, antibacterial, total antioxidant, scavenging, reducing power and ion chelating activities of green synthesized silver, copper and titanium dioxide nanoparticles using Artemisia haussknechtii leaf extract. Artificial cells, nanomedicine, and biotechnology 46: 2066-2081.
- Jafri L, et al. (2017) Invitro assessment of antioxidant potential and determination of polyphenolic compounds of Hedera nepalensis K. Koch. Arabian Journal of Chemistry 10: S3699- S3706.
- Naz S , et al. (2018) Anti cancer and antibacterial potential of Rhus punjabensis and CuO nanoparticles. Natural product research 1-6.
- Javed R, et al. (2017) PVP and PEG doped CuO nanoparticles are more biologically active: Antibacterial, antioxidant, antidiabetic and cytotoxic perspective. Materials Science and Engineering: C 79: 108-115.
- Fatima H, et al. (2015) Extraction optimization of medicinally important metabolites from Datura innoxia Mill.: an in vitro biological and phytochemical investigation. BMC complementary and alternative medicine 15: 376.
- Almajano MP, et al. (2008) Antioxidant and antimicrobial activities of tea infusions. Food chemistry108: 55-63.
- Astill C, et al. (2001) Factors affecting the caffeine and polyphenol contents of black and greentea infusions. Journal of agricultural and food chemistry 49: 5340-5347.
- Khalid S, et al. (2019) Calixarene coated gold nanoparticles as a novel therapeutic agent. Arabian Journal of Chemistry.
- Phull AR, et al. (2016) Antioxidant, cytotoxic and antimicrobial activities of green synthesized silver nanoparticles from the crude extract of Bergenia Ciliata. Future Journal of Pharmaceutical Sciences 2: 31-36.
- NIKAVAR B and N Yousefian (2009) Inhibitory effects of six Allium species on α-amylase enzyme activity.
- Lalitha A, R Subbaiya and P Ponmurugan (2013) Green synthesis of silver nanoparticles from leaf extract Azadirachta indica and to study its anti-bacterial and antioxidant property. Int J Curr Microbiol App Sci, 2: 228-235.
- Saif S, et al. (2016) Plant mediated green synthesis of CuO nanoparticles: comparison of toxicity of engineered and plant-mediated CuO nanoparticles towards Daphnia Magna. Nanomaterials 6: 205. 9 J
- Ghasemzadeh A and N Ghasemzadeh (2011) Flavonoids and phenolic acids: Role and biochemical activity in plants and humans. Journal of medicinal plants research 5: 6697-6703.
- Liao Kl and Mc Yin (2000) Individual and combined antioxidant effects of seven phenolic agents in human erythrocyte membrane ghosts and phosphatidylcholine liposomes systems: the importance of the partition coefficient. Journal of agricultural and food chemistry 48: 2266-2270.
- Asamoah RB, et al. (2020) Synthesis and characterization of zinc and copper oxide nanoparticles and their antibacterial activity. Beilstein Archives 12.
- Karthik L, et al. (2013) Environmental and human impact on marine microorganisms synthesized nanoparticles. Marine biomaterials: characterization, isolation, and applications.CRC Press, Boca Raton 253-272.
- Ghorbani HR, I Fazeli and AA Fallahi (2015) Biosynthesis of copper oxide nanoparticles using an extract of E. coli. Oriental Journal of Chemistry 31: 515-517.
- Nethravathi P, et al. (2015) Tinospora cordifolia mediated facile green synthesis of cupric oxide nanoparticles and their photocatalytic, antioxidant and antibacterial properties. Materials Science in Semiconductor Processing, 33: 81-88.
- Arun L, et al. (2018) Effect of Ni 2+ doping on chemocatalytic and super capacitor performance of biosynthesized nanostructured CuO. Journal of Materials Science: Materials in Electronics 29: 21180-21193.
- Mosleh S, et al. (2018) Sonochemical-assisted synthesis of CuO/Cu2O/Cu nanoparticles as an efficient photocatalyst for simultaneous degradation of pollutant dyes in a rotating packed bed reactor: LED illumination and central composite design optimization. Ultrasonics sonochemistry 40: 601-610.
- Mondal K (2017) Recent advances in the synthesis of metal oxide nanofibers and their environmental remediation applications. Inventions 2: 9.
- Khashan K, M Jabir and F Abdul Ameer (2018) Preparation and characterization of copper oxide nanoparticles decorated carbon nanoparticles using laser ablation in liquid. in Journal of Physics: Conference Series. IOP Publishing.
- Luna IZ and BAE Commission (2015) Preparation and characterization of copper oxide nanoparticles synthesized via chemical precipitation method. Open Access Library Journal 2: 1.
- Dahrul M and H Alatas (2016) Preparation, and optical properties study of CuO thin film as an applied solar cell on LAPAN-IPB Satellite. Procedia Environmental Sciences 33: 661-667.
- Wang Z and P An (2017) Characterization Of Copper Complex Nanoparticles Synthesized By Plant Polyphenols. BioRxiv 134940.
- Radha krishnan AA and BB Beena (2014) Structural and opticalabsorption analysis of CuO nanoparticles. Indian Journal of Advances in Chemical Science 2: 158-161.
- Shahmiri M, et al. (2013) Preparation of PVP-coated copper oxide nanosheets as antibacterial and antifungal agents. Journal of Materials Research 28: 3109-3118.
- Priya RS, D Geetha and P Ramesh (2016) Antioxidant activity of chemically synthesized AgNPs and biosynthesized Pongamia pinnata leaf extract mediated AgNPs–A comparative study. Ecotoxicology and environmental safety 134: 308-318.
- Chung IM, et al. (2019) Impact of Copper Oxide Nanoparticles on Enhancement of Bioactive Compounds Using Cell Suspension Cultures of Gymnema Sylvestre (Retz.) R. Br. Applied Sciences 9: 2165.
- Thiruvengadam M, et al. (2019) Synthesis, characterization and pharmacological potential of green synthesized copper nanoparticles. Bioprocess and biosystems engineering 42: 1769-1777.
- Kumar VV and SP Anthony (2016) Antimicrobial studies of metal and metal oxide nanoparticles, in Surface chemistry of nanomaterials. Elsevier 265-300.
- Chen J, et al. (2019) Various antibacterial mechanisms of biosynthesized copper oxide nanoparticles against soilborne Ralstonia solanacearum. RSC advances 9: 3788-3799.
- Nabila MI and K Kannabiran (2018) Biosynthesis, characterization and antibacterial activity of copper oxide nanoparticles (CuO NPs) from actinomycetes. Biocatalysis and agricultural biotechnology 15: 56-62.
- Naz S, A Gul and M Zia (2019) Toxicity of copper oxide nanoparticles: a review study. IET nanobiotechnology.
- Niño-Martínez N, et al. (2019) Molecular mechanisms of bacterial resistance to metal and metal oxide nanoparticles. International journal of molecular sciences 20: 2808.
- Azam A, et al. (2012) Antimicrobial activity of metal oxide nanoparticles against Gram-positive and Gram-negative bacteria: a comparative study. International journal of nanomedicine 7: 6003.
- Ren G, et al. (2009) Characterisation of copperoxide nanoparticles for antimicrobial applications. International journal of antimicrobial agents 33: 587-590.
- Vasantharaj S, et al. (2019) Synthesis of eco-friendly copper oxide nanoparticles for fabrication over textile fabrics: characterization of antibacterial activity and dye degradation potential. Journal of Photochemistry and Photobiology B: Biology 191: 143-149.
- Naika HR, et al. (2015) Green synthesis of CuO nanoparticles using Gloriosa superba L. extract and their antibacterial activity. Journal of Taibah University for Science 9: 7-12.
- Naz S, et al. (2018) Low-temperature synthesis of hierarchical structures of copper oxide and their superior biological activity. IET nanobiotechnology12: 968-972.
- Wei Y, et al. (2010) Synthesis of stable, low-dispersity copper nanoparticles and nanorods and their antifungal and catalytic properties. The Journal of Physical Chemistry C 114: 15612- 15616.
- Amiri M, et al. (2017) Antimicrobialeffectofcopperoxidenanoparticlesonsomeoralbacteriaand candida species. Journal of dental biomaterials 4: 347.
- Ates M, et al. (2013) Comparative evaluation of the impact of Zn and ZnO nanoparticles on brine shrimp (Artemia salina) larvae: effects of particle size and solubility on toxicity. Environmental Science: Processes & Impacts 15: 225-233.
Figures at a glance